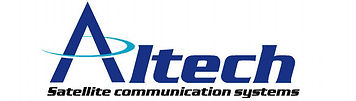
3.2.1 Current Atmospheric Effects Models
Introduction
This section covers the main models of the atmospheric effects on satellite signals propagation, and provides an understanding of their use and rationale. These methods have been tested based on the available data collected by researchers and have been shown compatible accuracy with the natural variability of propagation phenomena and adequate for present applications in system planning. However, there is insufficient data to support these models for frequencies above 40 GHz, and the models only provide a theoretical approach combined with empirical interpolations from data available from lower frequencies not in the V/W band. Throughout this literature review, more detailed explanation is given for the main atmospheric effects while secondary effects are discussed briefly.
An electromagnetic wave propagating between an earth station and a satellite must pass through the earth’s atmosphere, including the ionosphere. During this propagation, losses caused by the earth’s atmosphere will occur as a result of two phenomena: (1) energy absorption by atmospheric gases, and (2) adverse weather conditions. To distinguish between these, the weather-related losses are referred to as atmospheric attenuation and the absorption losses simply as atmospheric absorption [1].
The International Telecommunications Union (ITU) describes the following parameters as the key atmospheric effects above 1 GHz, and for low elevation angles [2]:
- absorption in atmospheric gases; absorption, scattering and depolarization by hydrometeors (water and ice droplets in precipitation, clouds, etc.); and emission noise from absorbing media; all of which are especially important at frequencies above about 10 GHz;
- loss of signal due to beam-divergence of the earth-station antenna, due to the normal refraction in the atmosphere;
- a decrease in effective antenna gain, due to phase decorrelation across the antenna aperture, caused by irregularities in the refractive-index structure;
- relatively slow fading due to beam-bending caused by large-scale changes in refractive index; more rapid fading (scintillation) and variations in angle of arrival, due to small-scale variations in refractive index;
- possible limitations in bandwidth due to multiple scattering or multipath effects, especially in high-capacity digital systems;
- attenuation by the local environment of the ground terminal (buildings, trees, etc.).
The main atmospheric absorption effects are caused by atmospheric gases, clouds, and fog; and the main atmospheric attenuation is caused by rain. Additionally, fading due to scintillation also contributes to propagations losses, as well as depolarization, which is caused by free electrons in the ionosphere, but also rain and ice [1]. Since the onset of interest in satellite communications, several research projects contributed to the development of the modern attenuation models. The earliest work comprised the comparison of experimental measurements with quantum mechanics theories, which were unified with the modern theoretical and experimental knowledge of the millimeter wave propagation characteristics in satellite communications.
Gaseous Attenuation Model
Main Models:
Liebe Complex Refractivity Model
Model presented by the ITU-R P.676-3
Description:
Recommendation ITU-R P.676 provides the modern method to estimate attenuation caused by atmospheric gases in satellite communications. For the 1-1000 GHz range, this model estimates gaseous attenuation by computing the summation of individual gaseous absorption lines. For the 1-350 GHz frequency range, a simplified approximate method to estimate gaseous attenuation can be used [2].
The specific attenuation at frequencies up to 1000 GHz can be obtained with the most accuracy for any value of pressure, temperature and humidity by means of a summation of the individual resonance lines from oxygen and water vapor, together with small additional factors for the spectrum of oxygen below 10 GHz, pressure-induced nitrogen attenuation and the excess water vapor absorption found experimentally [3].
The specific gaseous attenuation is given by Eq. 1 [2]:
3.2 Physical Models







where the o and w subscripts are the specific attenuation (dB/km) due to dry air and water vapor, respectively, f is the frequency (GHz), and N(f) is the imaginary part of the frequency-dependent complex refractivity [2]:
The mean attenuation of gases on earth-space paths in the 10 to 100 GHz frequency range has been theoretically modeled and experimentally measured. Above 20 GHz, gaseous absorption can have a significant effect on a communication system design depending on the specific frequency of operation because some frequencies have irregular behaviors. Because of the large frequency dependence of the gaseous absorption, an earth-space communication system designer should avoid the high absorption frequency bands. Alternatively, designers of secure short-haul terrestrial systems can utilize these high attenuation frequency bands to provide system isolation. In the frequency range from 10 to 100 GHz the water vapor absorption band centered at 22.235 GHz and the oxygen absorption lines extending from 53.5 to 65.2 GHz are the only significant contributors to gaseous attenuation. The next higher frequency absorption bands occur at a frequency of 118.8 GHz due to oxygen and water vapor [4].
The development of the current model started with the pioneering work in [5], which first used quantum mechanics to describe the atmospheric millimeter spectra and compared it with experimental results. The first measurements were obtained by calculating the attenuation of a signal in dry air in comparison to moist air, and also by using an interesting method involving the temperature of the antennas with respect to the absorption rate. However, the most accurate method at the time was the cavity method, in which the parameters of a cavity fed by microwaves were influenced by changing the moisture content of the air in the cavity [4]. The following steps were: the advances in the spectroscopic data compared to the models of the microwave spectrum [6 ,7 ,8 ]; line shape theory [9], measurements of refraction [10], modeling, reports of abnormal windows of absorption, and collection of data to support an empirical vapor continuum [11]. Therefore, a unified model of those advancements was first unified in [12] and provides the foundation of the modern attenuation model.
Ionospheric Effects Models
The ionosphere generally has a minor effect on the propagation of radio waves in the V/W band, which include scintillation, gas absorption, angle of arrival, delay, and depolarization. However, the density of electrons in the ionosphere varies as a function of geomagnetic latitude, diurnal cycle, yearly cycle, and solar cycle. Fortunately, most U.S. ground station-satellite paths pass through the midlatitude electron density region, which is the most homogeneous region.
Tropospheric Delays Models
Any satellite system that requires high accuracy such as range-rate and position-location systems will need to mitigate the propagation delay effect caused by the troposphere. For example, extremely high switching rate TDMA systems require these corrections. The effects arise primarily due to the oxygen and water vapor in the lower troposphere. Researchers have measured that total propagation delay errors in the order of 8 nanoseconds. Estimation techniques, based on the measurement of the surface pressure, temperature and relative humidity have been which can readily reduce this error to less than 1 nanosecond. Models are based on measurements of the tropospheric refractivity compared to meteorological data from widely separated locations, which were then used to support mathematical predictions [14].
Clouds and Fog Attenuation Models
Main Models:
The ITU-R Cloud Attenuation Model
Slobin Cloud Model
Altshuler Model
Description:
The attenuation due to clouds may be a factor of importance especially for the V/W Band, and any RF system operating above 10 GHz. The water droplets that form clouds and fog are generally smaller than about .01 cm in diameter. This allows the Rayleigh approximation to be used to calculate specific attenuation in clouds and fog for frequencies in the V/W band. Using this approximation, the specific attenuation is modeled by clouds or fog with Eq. 3 [15 ]:
where:
Kl : specific attenuation coefficient ((dB/km)/(g/m3));
M : liquid water density in the cloud or fog (g/m3).
A mathematical model based on Rayleigh scattering, which uses a double-Debye model for the dielectric permittivity of water, can be used to calculate the value of Kl for frequencies up to 1000 GHz in Eq. 4 [15]:
where f is the frequency (GHz), and epsilon is the complex dielectric permittivity of water.
To obtain the attenuation due to clouds for a given probability, the statistics of the total columnar content of liquid water (kg/m2), or an equivalent number, must be known and is expressed by Eq. 5[15]:
where theta is the elevation angle, L is the total columnar content of liquid water, and the specific attenuation coefficient Kl is read from [15].
The total columnar content of cloud liquid can be obtained from radiosonde and radiometric measurements. Radiosonde data is available on the Internet; however, it has limited time resolution and is applicable to zenith paths exclusivly. The total columnar content of cloud liquid water can be retrieved from radiometric measurements at appropriate frequencies along a specific path. Annual values of total columnar content of cloud liquid water, L (kg/m2) are recorded and distributed in the form of digital maps in the Radiocommunication Study Group 3 website [15].
A relatively simple procedure for the estimation of fog attenuation from fog density or fog visibility data has been developed based on regression analysis performed on a large set of fog attenuation data over a wide range of frequencies (10 to 100 GHz) and temperatures (-8 to 25EC), using tabulated values of indices of refraction. This model predicts attenuation by correlating [16 ]:
- Normalized fog attenuation
- Frequency
- Temperature
- Fog density
- Fog extent
Unfortunately, the fog density is a value difficult to obtain, and can vary greatly within small intervals of time and location. The standard error of the estimation procedure described above is 0.14 db. The procedure should not be used for frequencies below 30GHz, since the error is comparable in magnitude to the fog attenuation itself [16].
Rain Attenuation
Main Models:
ITU Rain Model; tested below 40 GHz
Crane Global Model; tested below 40 GHz
Crane Two-Component Model; tested below 40 GHz
DAH Model; applicable from 4 to 35 GHz
ExCell Rain Attenuation Model ; tested below 40 GHz
Manning Model
Description:
Models under 55 GHz
These models are generally accepted below 55 GHz, and their validity beyond 35 GHz has been questioned by researchers [17]. Two general approaches have been used to calculate the rain attenuation A: (1) a "theoretical method employing a uniformly random distribution of raindrops modeled as water spheres or more complex shapes," and (2) an "empirical procedure based on the approximate relation between the specific attenuation, and the rain rate R (mm/h)" in Eq.6 [18 ].
where k and alpha are functions of frequency and rain temperature, Ls is the slant path length through the rain, and AF is an adjustment factor determined by regretion analysis. Values for the coefficients k and in the range from 1 to 1000 GHz have been developed from curve-fitting to power-law coefficients derived from scattering calculations. Values for the constant coefficients k and are different for signals with different polarization. For horizontally polarized signals, kH and alpahaH are used, while kV and alphaV are used for vertical polarization [19 ]. For linear and circular polarization, and all path geometries, the coefficients in Eq. 6 are determined by Eq. 7 and Eq. 8:
where theta is the path elevation angle and t is the polarization tilt angle relative to the horizontal (t = 45° for circular polarization).
Rain Attenuation Models for the V/W Band
In the literature, different methodologies are used for rain attenuation time-series synthesis for extremely high frequency (EHF) (part of the V/W Band) including the spectral model, synthetic storm techniques, the two-sample model, and N-state Markov chain models. These models have been developed by researchers through the analysis of empirical measurements performed during scientific satellite experiments in the V band. Most of these models cannot be used for W band rain attenuation because they need data from empirical measurements as input, and no attenuation record database is available for the W-band satellite link [20].
According to the experiments in [18], N-state Markov chain model is considered one of the best choices to achomplish some credible theoretical attenuation calculations. The input data requested to run the model are the geometrical configuration (satellite and Earth station locations), radiowave characteristics (frequency and polarization), and the climatological parameters given by ITU-R radio-meteorological maps [21]. Moreover, some preliminary designs of digital broadcasting use this method as their basis for rain attenuation predictions [22].
Depolarization
Main models:
Chu Empirical Models
ITU-R Model
Description:
Rain depolarization can be modeled using techniques similar to those applied to rain attenuation. The essential difference is that the raindrops are assumed to be oblate spheroids, and the key parameters are: (1) specific attenuation of power flux density of wave, (2) specific phase lag of wave, (3) orientation, (3) frequency, and (4) elevation angle. These transmission coefficients have been calculated by several researchers as a function of rain rate by cross polarization discrimination [16].
The Chu model employs a Gausian model to account for the canting angle variations. The key parameters of his model are:
- Rain attenuation
- Tilt angle of linearly polarized electric field vector
- Frequency
- Elevation angle
Chu derived a "semi-empirical formula for depolarization versus attenuation that agrees with experimental results over a wide range of frequency, polarization tilt angle and elevation angle" [16]. The ITU method uses the similar parameters to the Chu model, and applies below 55 GHz [2].
Scintillation Model
Main Models:
Karasawa Model
ITU-R Model
Description:
Rain attenuation, as mentioned already, is expected to be the predominant attenuation in the V/W Band. However, "for the design of low margin systems, especially those at high frequencies and low elevation angles, scintillation effects must be accounted for to design a link budget accurately" [23 ].
The ITU-R model is based on the Karasawa model. This model quantifies scintillation in terms of the "instantaneous signal amplitude, the standard deviation of scintillation intensity, a Gaussian random process that describes the short-term behavior of scintillation, and a gamma density function that describes the long-term changes in scintillation intensity. The scintillation intensity comes from the dependence of scintillation on environmental and system parameters, and it varies as a function of temperature and atmospheric water vapor concentration. Scintillation is also a function of elevation angle, signal frequency (weak), and path length. " In general, although really not as significant as rain for the V/W band, the scintillation can be calculated from the antenna size and efficiency, the antenna elevation angle, the RF frequency, the average local monthly temperature, and the average local monthly relative humidity [16] [24].
References
[1] D. Roddy, “Radio Wave Propagation,” in Satellite Communications, U.S.A., McGraw-Hill, 2001, pp. 91
[2] ITU-R, " Propagation data and prediction methods required for the design of Earth-space telecommunication systems", Recommendations and Reports of the ITU-R, Report P.618-10-2, International Telecommunications Union, Geneva, Switzerland, 2009.
[3] ITU-R, "Attenuation by Atmospheric Gases", Recommendations and Reports of the ITU-R, Report 719-2, Vol. V (Propagation in Non-ionised Media), International Telecommunications Union, Geneva, Switzerland, 1994.
[4] Ippolito, Louis J., “Propagation effects handbook for satellite systems design. A summary of propagation impairments on 10 to 100 GHz satellite links with techniques for system design”, The Smithsonian/NASA Astrophysics Data System, Chapter IV, pp. 1-25.
[5] Van Vleck, J. H., “The absorption of microwaves by oxygen and uncondensed water vapor”, in Phys. Rev., Volume 7, pp. 413-433
[6] Liebe, H. J., G. G. Gimmestad, and J. D. Hopponen, “Atmospheric oxygen microwave spectrum--Experiment versus theory”, In Trans. Antennas Propag., AP-25(3), 327-335, 1977.
[7] Poynter, R. L., and H. M. Pickett, Submillimeter, mil-limeter, and microwave spectral line catalogue, JPL Publ. 80-23, Jet Propul. Lab.,NASA, Pasadena, Calif., June, 1980.
[8] Rothman, L. S., AFGL atmospheric absorption line parameters compilation: 1980 version, Appl. Opt. 20(5), 791-795, 1981.
[9] Rosenkranz, P. W. (1975), “Shape of the 5 mm oxygen band in the atmosphere”, IEEE Trans. Antennas Propag., AP-23(4), 498-506.
[10]Kemp, A. J., J. R. Birch, and M. N. Afsar , The refractive index of water vapor: A comparison of measurement and theory, Infrared Phys.,
18, 827-833, 1978
[11] Crane, R. (1980), Attenuation estimates for millimeter wave windows near 94, 140 and 220 GHz, ERT Doc. P-A502, Environ. Res. & Technol., Inc., Concord, Mass., May
[12] Liebe HJ, “Modeling attenuation and phase of radio waves in air at frequencies below 1000 GHz”, Radio Science, 16:183–1199, 1983
[14]Hopfield, H.S., “Tropospheric Effect on Electromagnetically Measure Range: Prediction from Surface Weather Data,” Radio Science, 1971, Vol. 6, pp. 357-367.
[15] ITU-R, "Attenuation due to clouds and fog", Recommendations and Reports of the ITU-R, Report 840-5, International Telecommunications Union, Geneva, Switzerland, 2012.
[16] Ippolito, L, “Propagation Effects Handbook for Satellite Systems Design,” Stanford Telecom ACS, prepared for Jet Propulsion Laboratory, Pasadena, CA, 1998.
[17] Brost, G.A.; Cook, W.G.; , "Analysis of empirical rain attenuation models for satellite communications at Q to W band frequencies,"Antennas and Propagation (EUCAP), 2012 6th European Conference on , vol., no., pp.1455-1459, 26-30 March 2012
[18] Olsen, R. L., D. V. Roger, and D. B. Hodge, "The aRb relation in the calculation of rain attenuation," IEEE Trans. Antenna and Propagation, Vol. 26, 1978.
[19] ITU-R, "Specific attenuation model for rain for use in prediction models", Recommendations and Reports of the ITU-R, Report 840-5, International Telecommunications Union, Geneva, Switzerland, 2012.
[20] Sacchi, C.; Rossi, T.; Ruggieri, M.; Granelli, F.; , "Efficient Waveform Design for High-Bit-Rate W-band Satellite Transmissions,"Aerospace and Electronic Systems, IEEE Transactions on , vol.47, no.2, pp.974-995, April 2011
[21] ITU-R Recommendation, “Prediction method of fade dynamics on Earth-space path.” Geneva (CH), Mar. 2005.
[22]Fantinato, A.; Conci, N.; Rossi, T.; Sacchi, C.; , "Performance analysis of W-band satellite HDTV broadcasting," Aerospace Conference, 2011 IEEE , vol., no., pp.1-12, 5-12 March 2011
[23]Mayer, C.E.; Jaeger, B.E.; Crane, R.K.; Xuhe Wang; , "Ka-band scintillations: measurements and model predictions," Proceedings of the IEEE , vol.85, no.6, pp.936-945, Jun 1997
[24] Karasawa, Y., M. Yamada, and J. E. Allnutt (1988), A New Prediction Method for Tropospheric Scintillation in Satellite Communicationsî, IEEE Trans., AP-36, pp. 1608-1614